Gamma-Ray Bursts
A star exploded just 625 million years after the Big Bang, but the radiation of this event didn’t reach Earth until last spring. This gamma-ray burst was named GRB 090423. It is the most distant astronomical object yet discovered. Jochen Greiner and his colleagues at the Max Planck Institute for Extraterrestrial Physics in Garching investigate such cosmic ‘ignition sparks’ at the edge of space and time.
Text: Helmut Hornung

It must have been a violent catastrophe. Somewhere in the early universe, a star blew up – a heavyweight with several times the mass of our Sun. In the course of this detonation, within less than ten seconds, as much energy was released as the Sun has produced during its entire 10-billion-year lifetime. The radiation signal propagated away from the site of this inferno at the speed of light. After travelling for 13 billion years, the flash finally arrived near Earth: on April 23, 2009, it flared up in the Leo constellation – astronomers observed what is known as a gamma-ray burst, GRB 090423.
The first such flash was registered more than 40 years ago. On July 2, 1967, American Vela espionage satellites detected gamma rays that could not have originated from secret above-ground nuclear weapons tests that the orbiting spies were watching for. The measurements they recorded were not published until 1973 – with the conclusion that these signals must have come from the depths of the universe. In the years that followed, scientists investigated the gamma-ray burst phenomenon with instruments designed specifically for this purpose.
From 1991 until its controlled crash nine years later, the Compton space observatory registered about 2,000 gamma-ray flashes. The scientists learned that the gamma-ray bursts are randomly distributed and arrive from all directions without recognizably predominant sites of origin in the sky.
Gamma-ray bursts – signs of an intergalactic battle?
What is happening in space? Could this be “star wars”? Is it the detonation of bombs from highly developed civilizations? The distance to the sites of the gamma-ray bursts was long the subject of great controversy. It has now become clear that the bursts come from very far away, from distances of billions of light-years. A mechanism must thus be found to explain the immense energy generation, such as conversion of mass into radiation with incredibly high efficiency. Over the years, astrophysicists have put forward at least 150 different theories – most of which have since been dismissed.

The issue is further complicated by two sub-classes that were discovered through Compton measurements: gamma-ray bursts with a short duration of less than one to two seconds, and those that typically last between ten and one hundred seconds. The short events are possibly created during the fusion of two neutron stars that had been orbiting each other for some time; alternatively, such a remnant star might have an even more compact partner – a black hole whose attractive force it cannot resist, finally plunging into its gravitational sink.
Long gamma-ray bursts constitute by far the larger population. In the presently accepted scenario, they arise when a very massive star collapses at the end of its evolution, after the fuel for nuclear fusion in its core has run out and the reactor fizzles. As radiation pressure from the inside decreases, gravity wins and the star contracts. Finally, electrons and protons are so tightly compressed that they merge to form neutrons. This reduces the pressure in the core even further and finally the entire star collapses. Central density then reaches that of an atomic nucleus – about 100 million tons per cubic centimeter.
Scraps of a star disappear behind the event horizon
Scientists still do not know exactly how arrival at this density limit transforms the implosion into an explosion. The shockwave forming at this “wall” appears to be insufficient: neutrinos – electrically neutral elementary particles that hardly interact with other particles – play a role, as does the stellar rotation, which accelerates during the collapse and channels the collapsing flow of matter into a fast-rotating disk.

Moreover, a jet traveling at almost the speed of light is ejected from the two polar regions of the collapsed star. Within this jet of plasma, shockwaves are formed by collisions of faster particles with slower ones, and these, in turn, generate gamma rays. Deeper inside, the remains of the collapsing star disappear behind the event horizon of a cosmic mass swallower: a black hole is born.
Astrophysicists are working on the theoretical details of this scenario. What causes gas to stream into a central compact object at high velocity and thus convert its surroundings into a source of high-energy light and plasma jets? If you want to understand this, there is only one thing to do: keep a close eye on nature during such a process. This is where gamma-ray bursts offer an ideal starting point, because they represent the most direct messengers of such spectacular cosmic events.
There are two practical paths of progress: Gamma rays of the burst can be measured directly in order to infer the source and the emission mechanism from its time profile and energy spectrum. Alternatively, one can observe the afterglow of the eruption in the X-ray spectrum, and also in the visible and infrared spectra.
The prompt gamma-ray burst phase is measured through the gamma-ray eyes of the Swift, INTEGRAL and Fermi satellites; the afterglow is registered at lower wavelengths by instruments including those aboard Swift, as well as the GROND instrument at the Max Planck Society’s 2.2-meter telescope at La Silla in the Chilean Andes. GROND was developed entirely at the Max Planck Institute for Extraterrestrial Physics, and the detectors for one of the Fermi onboard instruments were contributed by the members of the high-energy astrophysics group at this institute.
Swift was launched into space in November 2004 and has since used its several telescopes to monitor the sky for gamma bursts. Its measurements can also detect gamma-ray burst afterglows. Scientists didn’t discover this until 1997, with the Italian BeppoSax satellite – 30 years after the incidental registration of the first burst of this type. This afterglow is believed to result from the interaction of the star’s outward-moving explosion front with surrounding matter, and can be observed for hours to days in a wide range of wavelengths, from X-ray, ultraviolet and optical radiation to radio waves.
Within one minute of detecting a new gamma-ray burst, Swift can turn its X-ray telescope to the direction determined in the sky. From measurements early in the mission, the scientists were surprised by the variations of that X-ray glow’s intensity: “Instead of the expected exponential decay, we find a much steeper decline within the first few minutes. During the first few hours, the emission often reaches a phase of constant intensity. Sometimes it then flares up again for about half an hour, during which the X-ray brightness changes by a factor of up to 100,” says Jochen Greiner from the Max Planck Institute for Extraterrestrial Physics. While the cause of the plateau phase is not known, Greiner and his colleagues take the sudden flaring as a sign of interactions within the explosion cloud.
The light is shared
Known as Fermi (originally GLAST for Gamma-ray Large Area Space Telescope), the most powerful gamma-ray observatory to date arrived at its observation post in its Earth orbit in July 2008. On board, the main telescope LAT (Large Area Telescope) and the GLAST Burst Monitor (GBM) with its 14 detectors share the incident gamma-ray light.

LAT scans the gamma-ray spectrum at high energies of between 20 mega-electronvolts and 300 giga-electronvolts. The GBM detectors developed at the Max Planck Institute for Extraterrestrial Physics, on the other hand, monitor radiation at lower energies of between 15 kilo-electronvolts and 1 mega-electronvolt, and between 150 kilo-electronvolts and 30 mega-electronvolts, respectively, for its two different detector types. The gamma-ray monitor has an omnidirectional field of view, and raises an alarm as soon as it detects a gamma-ray burst. The satellite with its main instrument then turns to the direction estimated from the GBM data.
The detection of gamma-rays with the LAT functions differently than with an optical telescope, because light of such high energy simply cannot be deflected into a focal point. Instead, the instrument uses the pair production effect: inside the detector, the massless light particle – the photon – transforms into a pair of oppositely charged massive particles – a negatively charged electron and its positively charged antiparticle, the positron. “With the aid of a tracking detector, we use the interaction of the charged particles with the individual detector units to determine their path, from which the initial energy and direction of the gamma-ray photon can be determined,” says Gottfried Kanbach from the Garching-based high-energy astrophysics group, explaining the principle they employ to take the measurements.

GBM houses 14 crystal detectors, 12 made of sodium iodide (NaI) and 2 made of bismuth germanate (BGO). These are scintillation crystals, so named because, upon the interaction of a gamma-ray photon, they produce a brief flash of optical light – they “scintillate.” Light-sensitive photo-multiplier tubes convert this scintillation light into an electrically usable signal. The central GBM board computer processes this signal to derive an initial and still crude arrival direction. “This is then transmitted to both the LAT instrument and the ground station, from where the burst alarm is reported to a large number of observatories worldwide,” says Max Planck scientist Andreas von Kienlin. The goal is to monitor the afterglow of a gamma-ray burst in a wide range of possible wavelengths.
Rising beyond the limits
Only recently was it possible to open one of these wavelength windows: “We are finally observing the afterglow in the infrared region systematically as well,” says Jochen Greiner. “I feel this marks a breakthrough in method because it allows the redshift to be determined directly from the gamma-ray burst signal – instead of later measuring the redshift of the galaxy where the explosion appeared.” Moreover, it expands the accessible redshift region to earlier cosmic times. Quasars, young galaxy cores that are so bright they can be seen at great distances, no longer mark the limits.

Determining the distance is of crucial importance in the study of gamma-ray bursts. As years have gone by, it has been possible to increasingly improve the sensitivity of detectors. Until recently, however, scientists monitored the gamma-ray burst afterglow mainly in the visible spectral band. This involved first recording images of the corresponding region in the sky in order to detect the exact position of the newly appearing fireball. The redshift was not measured until the following night with the aid of a spectrograph pointed precisely in that direction.
The GROND detector developed by Jochen Greiner radically improves this situation: “The instrument combines the detection of the afterglow with a rough determination of the distance. We can now determine the distance of a gamma-ray burst within minutes, or within one to two hours at worst, to within an accuracy of about 5 percent,” says the scientist. The successful new strategy has caught on: “During the last two years, GROND has enabled us to discover as many bursts at a redshift larger than four as we had discovered in ten years before.”
GROND is an abbreviation borrowed from Tolkien’s book Lord of the Rings and stands for Gamma-Ray burst Optical and Near-infrared Detector. GROND is basically a camera that can take measurements in visible and in infrared bands. The instrument, developed and built in collaboration with the Thuringian State Observatory in Tautenburg, achieves this using six beam splitters to divide the light into seven color channels. Each channel has its own detector, so that the seven channels are exposed simultaneously.
Swallowed by hydrogen
The distance to an object in the deep universe is determined from its cosmological redshift (see box on page 49). The so-called absorption edge of radiation from intergalactic hydrogen is an important marker. This occurs normally at a wavelength of 120 nanometers: radiation of shorter wavelengths is swallowed (absorbed) by the hydrogen atoms and the object disappears. The cosmological redshift moves this absorption edge to longer wavelengths. GROND allows this position of the shifted edge to be determined so that the recession velocity and thus the distance of the object can be derived.

Something that sounds so easy still has its technical difficulties – and this is why nowhere in the world is there another such instrument that operates in the visible and the near infrared spectra simultaneously. The infrared detectors must be cooled to a temperature of minus 210 degrees Celsius, for example. The optical detectors, on the other hand, must not be colder than minus 120 degrees Celsius, even though they are separated from the former by less than 10 centimeters. The lens system positioned in front of the infrared detectors must also be cooled to minus 200 degrees so as not to generate additional background radiation. Finally, exposures in the near infrared must typically be as short as only about 10 seconds – otherwise the detector will be dazzled by the bright, ever-present celestial background.
In order to record sources that are fainter than the normal celestial brightness in the near infrared, a telescope is usually pointed to a slightly offset area of the sky after each 10-second exposure. This is not possible with GROND, because its 45-second readout time of the optical detectors is much longer: that would entail pausing for 45 seconds every 10 seconds – an extremely inefficient observation method. “We have circumvented this problem with a small trick,” says Greiner. “Instead of moving the telescope, we internally move the image through a wobble mirror, like squinting in a circle as if we were rolling our eyes.”
GROND is one of three instruments installed at the 2.2-meter telescope owned by the Max Planck Society and the European Southern Observatory on the summit of La Silla in the Chilean Andes. GROND´s controlling computer is connected directly to the ground station of the Swift satellite. When it detects a gamma-ray burst during the Chilean night, GROND is activated without human intervention: within a short time, the current observation is interrupted, the telescope moves to the position transmitted by Swift, and a tilt mirror is placed into the beam path. Some two to five minutes after the alarm has gone off, GROND starts taking the first images. “In the case of GRB 090423, though, we had to wait 15 hours because the object had already set in the sky over La Silla,” recalls Greiner with regret.
Nevertheless, the observation of the burst of April 23 was a great success. The explosion of a high-mass star with a redshift of z = 8, dating it to about 13 billion years ago, is the most distant object astronomers have seen so far. With the finite speed of light, this means that, with increasing distance, they are advancing into ever earlier epochs of the universe. GRB 090423 also marks the earliest existence of a cosmic object ever observed.
The cycle of the elements is initiated
The first generation of stars, probably born a few hundred million years after the Big Bang out of gigantic clouds of gas, were composed mainly of hydrogen. In other words, they were very deficient in metals. Only nuclear fusion reactions inside these so-called Population III stars produced the first amounts of heavier elements, such as carbon, nitrogen and oxygen, and spread them into the vastness of space when they exploded. “This gave rise to new generations of stars, and the cosmic cycle of the elements started, with its ever increasing enrichment of heavy elements,” explains Max Planck scientist Roland Diehl. Our Sun, being a relatively metal-rich star, thus belongs to one of the later stellar generations. Did the star whose gamma-ray burst was registered on April 23 belong to this very first generation of stars, or to a somewhat later generation? A fascinating question, and one that remains to be answered. Just like the fundamental question: What was the universe made of at its beginnings?
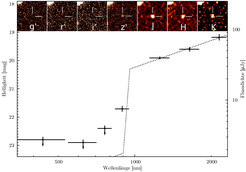
Gamma-ray bursts play an important role in the study of this question, since they light up their surroundings, and matter thus leaves behind characteristic fingerprints in the form of absorption lines in the spectra. Studying the abundances of metals in this very early time of the universe also promises valuable conclusions about details of star formation under the largely unknown conditions of that time.
Jochen Greiner points out yet another aspect: “The source of a gamma-ray burst is directly related to the formation of a black hole. What type of radiation just manages to escape such an extreme gravitational field as it is building up? What are the processes that cause matter to disappear behind the event horizon of such a compact object?” Gamma-ray light is the most energetic form of electromagnetic radiation and is thus very well suited to shed light on these processes.
So what is the next move for gamma-ray burst astronomy? The previous record holder was at a redshift of 6.7. It was detected by the Swift satellite in September 2008, and the distance was initially measured by GROND to within an accuracy of 5 percent. “The relatively short time from one redshift record to the next gives us hope that further distance records will be set in the near future,” says Greiner.
Observational cosmology is standing at a crossroads
The wide-ranging importance of gamma-ray bursts for observational cosmology is now definitely being recognized. After all, looking into earlier and earlier epochs of the universe is one of its main objectives. Extensive long-term observations, such as those with the Hubble Space Telescope, have been conducted and hundreds of nights with the telescopes of the 8-to-10-meter class have been spent in recent years on galaxies and on the nuclei of active galaxies in order to overcome the magic limit of redshift 7, which corresponds to a universe age of 780 million years. A 2.2-meter telescope has now been sufficient to make the leap. “This means,” says Jochen Greiner, “that observational cosmology is standing at a crossroads.”

Gamma-ray bursts may show how the very first “megastars” may have formed a further 400 to 500 million years earlier, at redshifts of 25 to 30. But the warm atmosphere of the Earth sets a redshift limit of about 13 that is difficult to overcome with today’s telescopes, and corresponds to a universe age of 330 million years. To look beyond, one would need either much larger telescopes or a new generation of gamma-ray satellites. Scientists at the Max Planck Institute for Extraterrestrial Physics proposed such a mission to the European Space Agency and the German Aerospace Center back in 2007. They now hope that the current measurements of GRB 090423 will give their proposal more weight – and that the sponsors may also succumb to the fascination of the chase for cosmic records.
GLOSSARY
Nuclear fusion
Stars are nothing more than huge nuclear fusion reactors. In the centers of these globes of gas, and in their explosions (supernovae), fusion processes occur at high pressure and temperatures of several million to several billion degrees, and atomic nuclei fuse to form new nuclei. Stars such as our Sun spend most of their lives fusing hydrogen into helium.
Black hole
An astronomical object whose gravitational attraction is so strong that not even radiation can escape. Black holes occur in two “weight classes”: as massive as stars, and millions of times more massive. Stellar black holes are created at the end of the stellar evolution of a massive star.
Spectrograph
This instrument – in the simplest case a prism, used by Isaac Newton in his experiments back in the 17th century – disperses light into its different wavelengths. Dark absorption lines appear in a color spectrum, with specific lines being able to characterize the source, allowing them to be attributed to specific chemical elements.
Stellar population
A term introduced by Walter Baade in 1944 for stars that are similar in terms of their composition (“metallicity”), their spatial distribution and their age. A distinction is made between three populations: the first stars are called Population III, while those that formed later but relatively early in a galaxy’s evolution are said to belong to Population II, and younger stars that formed in more mature epochs of galaxy evolution – such as the Sun – are called Population I.
The blue luminescence
Two other telescope installations in which the Max Planck Society has a stake through its Institute for Nuclear Physics in Heidelberg and its Institute of Physics in Munich also have the gamma sky in their sights: H.E.S.S. and MAGIC. But neither of them measures gamma rays directly. The Earth’s atmosphere is not transparent to this extremely energy-rich light – which is fortunate for life on Earth. Instead, these instruments register what is known as Cherenkov radiation. This radiation flash is generated when a particle shower caused by incident gamma rays in the upper atmosphere propagates at a velocity exceeding the local speed of light in that medium.
The initial interaction produces pairs – an electron and its antiparticle, the positron – which propagate in the atmosphere and are deflected in the electric fields of atomic nuclei. This generates secondary gamma-ray photons, which in turn produce new electron-positron pairs upon passing an atomic nucleus. A shower of high-velocity particles is thus generated in a cascade. The collective radiation from polarized atoms of the atmosphere as they experience this electromagnetic particle shower emerges as blue light – the Cherenkov radiation – for several billionths of a second at an altitude of around eight kilometers. It is precisely this blue light that is recorded by H.E.S.S. (High Energy Stereoscopic System) and MAGIC (Major Atmospheric Gamma-ray Imaging Cherenkov telescope).
Astronomical targets of the installations include the center of the Milky Way, rapidly rotating neutron stars (pulsars) and the remnants of supernovae. The two MAGIC telescopes at La Palma on the Canary Islands (photo) also specifically target gamma-ray bursts with the “stereoscopic view” of the atmosphere through their 17-meter mirrors; H.E.S.S. in South Africa is composed of four mirrors, and also targets such events when a satellite raises an alarm and the installation’s sky visibility renders it active.
Like raisins in rising dough
Cosmological redshift is a measure of the distance of an object. Galaxies virtually swim in space-time, which expands with the expansion of the universe.
Galaxies are carried along within, just like raisins in rising dough. This motion is imposed onto any electromagnetic radiation moving through the universe: if space-time increases by a certain factor as the radiation propagates, this is reflected in a corresponding increase in the radiation wavelength, resulting in a shift toward the redder wavelength region of the spectrum.
The redshift (abbreviated z) is defined as the ratio of the observed wavelength to the wavelength of the corresponding emission process at rest in the laboratory. Astronomers use redshift to measure the distance of the object and thus the point in time when the object emitted its radiation. Accordingly, z = 1 corresponds to a distance of 5.9 billion light-years; the universe was just under half its present age of 13.7 billion years when that radiation was emitted.